Wind Energy Output Calculations
This post contains affiliate links. I may earn a commission at no extra cost to you if you make a purchase. Note that I’m not a health or outdoor safety professional, so further research is advised. Your support keeps Outdoors A-Z running—thank you! Read the full disclosure.. Read the full disclosure here.
Wind energy stands at the forefront of renewable energy solutions, harnessing the power of the wind to generate electricity. As the world strives for sustainable and eco-friendly alternatives to traditional fossil fuels, wind energy has emerged as a promising contender, offering a clean, renewable, and abundant source of power. In the pursuit of maximizing the potential of wind energy, understanding how to calculate its output is paramount.
This blog post delves into the intricate world of wind energy output calculations, unraveling the complexities behind the seemingly simple act of harnessing the wind. From the physics governing wind energy generation to the real-world factors that influence its output, we will explore the methodologies, formulas, and considerations essential for accurate predictions.
Table of Contents
Basics of Wind Energy
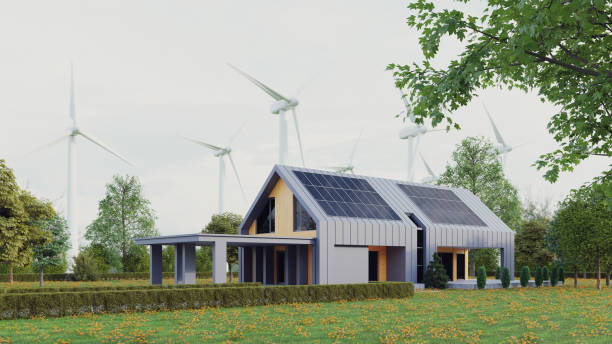
Wind energy, often referred to as wind power, is the process of converting the kinetic energy from wind into mechanical power or electricity. This conversion is achieved through the use of wind turbines, which consist of blades mounted on a rotor. As the wind flows over these blades, it causes them to rotate, driving a generator to produce electricity. This ingenious process capitalizes on a natural phenomenon—wind—that has been harnessed by humans for centuries, evolving from simple windmills to advanced wind turbine technology.
Components of a Wind Energy System
A typical wind energy system comprises several key components:
- Wind Turbines: These are the iconic structures with large rotating blades seen in wind farms. Modern turbines come in various sizes and designs, but they all serve the same purpose: capturing wind energy.
- Blades: Blades are the most visible part of a wind turbine. Their design is crucial, as it affects the turbine’s efficiency in harnessing wind energy. Aerodynamic considerations and materials play a significant role in blade design.
- Nacelle: The nacelle houses the turbine’s critical components, including the generator, gearbox, and control systems. It is usually located atop the tower and rotates to face the wind for optimal energy capture.
- Tower: The tower supports the entire wind turbine, raising it high above the ground where wind speeds are higher and more consistent. Taller towers allow turbines to capture energy from higher altitudes.
Factors Influencing Wind Energy Output
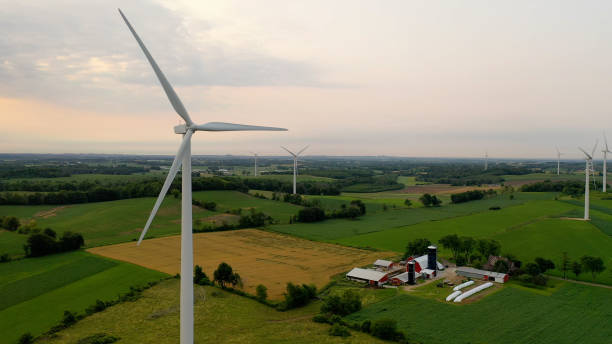
Several factors influence the output of a wind energy system, making it a dynamic and intricate field of study:
- Wind Speed: The most fundamental factor, wind speed, is directly related to the amount of energy a turbine can capture. Higher wind speeds result in more kinetic energy, leading to increased electricity generation.
- Turbine Efficiency: Turbines are designed with specific efficiency levels. The efficiency is influenced by the turbine’s size, blade design, and internal components. Understanding and optimizing these factors are crucial for maximizing energy output.
- Wind Direction: Turbines are designed to orient themselves into the wind. Changes in wind direction require the turbine to rotate, adjusting its position to maintain optimal wind capture.
In the next section, we will delve deeper into the fundamental formula used for wind energy output calculations, breaking down the variables and their significance in the pursuit of harnessing wind power efficiently and sustainably.
Wind Energy Output Calculation Formula
At the heart of predicting wind energy output lies a fundamental formula:
Output=0.5×A×ρ×V3×Cp
Let’s break down this formula to understand its components and their roles in determining the energy output of a wind turbine:
1. Area (A):
- Definition: The swept area or the circular area covered by the rotating blades.
- Significance: Larger turbines with longer blades have a greater swept area, allowing them to capture more wind energy.
2. Air Density (ρ):
- Definition: The mass of air per unit volume. It varies with altitude and temperature.
- Significance: Higher air density, typically found at lower altitudes and cooler temperatures, means there is more mass available to capture kinetic energy from the wind.
3. Wind Speed (V):
- Definition: The speed of the wind, typically measured at hub height where the turbine blades are located.
- Significance: Wind speed is a crucial factor; the energy output is proportional to the cube of the wind speed. This means even a small increase in wind speed significantly impacts the energy a turbine can capture.
4. Power Coefficient (Cp):
- Definition: A dimensionless factor representing the efficiency of the turbine in capturing the available wind energy.
- Significance: Cp value ranges between 0 and 1. A higher Cp indicates a more efficient turbine design, maximizing the conversion of wind energy into mechanical or electrical power.
Understanding and optimizing these variables are essential for accurate wind energy output predictions. Variations in any of these factors can significantly affect the overall energy production of a wind turbine. Engineers and researchers continuously work to improve each variable to enhance the efficiency and sustainability of wind energy systems.
Calculating Wind Speed
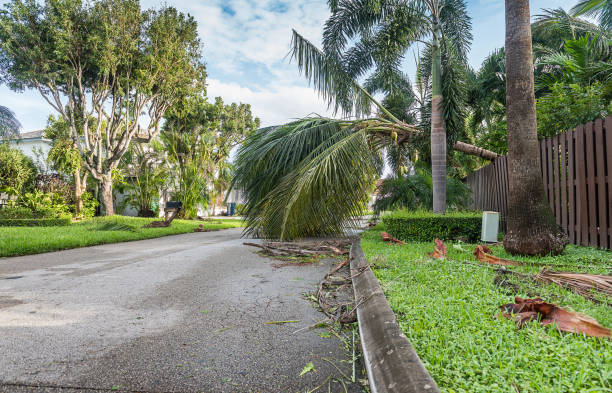
Wind speed is the primary driver of wind energy production. The kinetic energy available in the wind increases significantly with even small changes in speed, making accurate wind speed measurement critical for predicting energy output. Meteorological instruments called anemometers are commonly used to measure wind speed. These devices come in various types, including cup anemometers, vane anemometers, and sonic anemometers, each with its specific method of measurement.
Methods to Measure Wind Speed:
- Cup Anemometers: Cup anemometers consist of three or four cups attached to horizontal arms. As the wind blows, the cups rotate, and the speed of rotation is directly proportional to the wind speed. This method has been widely used for decades and provides reliable measurements.
- Vane Anemometers: Vane anemometers use a rotating vane or blade that aligns itself with the wind direction. The speed of rotation indicates wind speed. While these anemometers are simple and inexpensive, they are sensitive to wind direction changes.
- Sonic Anemometers: Sonic anemometers employ ultrasonic signals to measure wind speed and direction. These devices offer high accuracy and are commonly used in research applications. They are capable of providing real-time data and are not affected by mechanical parts’ wear and tear.
Impact of Wind Speed Variations on Energy Output:
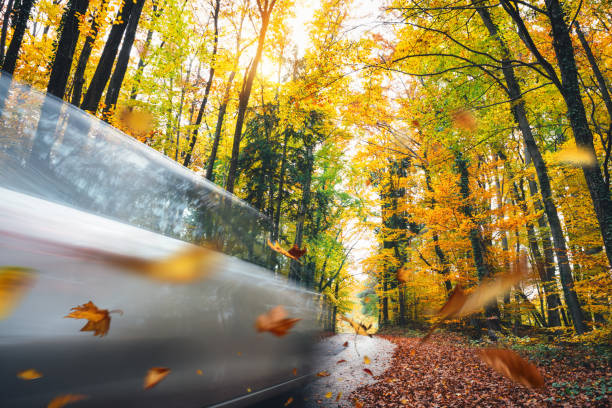
Small changes in wind speed have a profound impact on energy output due to the cube relationship in the power equation. For instance, doubling the wind speed results in eight times more power available for capture. Therefore, accurate measurement and prediction of wind speeds are crucial for optimizing wind energy production. Meteorological data, historical wind speed records, and advanced wind resource assessment tools are utilized to estimate and analyze wind speeds for wind energy projects.
Calculating Turbine Efficiency
Turbine efficiency, represented by the power coefficient (Cp), is a pivotal factor in determining how effectively a wind turbine converts the kinetic energy in the wind into mechanical or electrical power. A high Cp signifies an efficient turbine, capable of capturing a substantial portion of the available wind energy.
Definition of Power Coefficient (Cp):
The power coefficient (Cp) is a dimensionless number that represents the fraction of the total power in the wind that can be converted into mechanical power by the turbine. It is a crucial parameter in the wind energy output calculation formula. Mathematically, Cp is expressed as:
Cp=Power Extracted by the Turbine/Available Wind Power
An ideal wind turbine would have a Cp value of 0.59, known as the Betz limit. This theoretical limit, derived from aerodynamic principles, signifies the maximum amount of kinetic energy a wind turbine can capture from the wind. Real-world turbines, however, operate slightly below this limit due to practical constraints and aerodynamic losses.
Factors Affecting Turbine Efficiency:
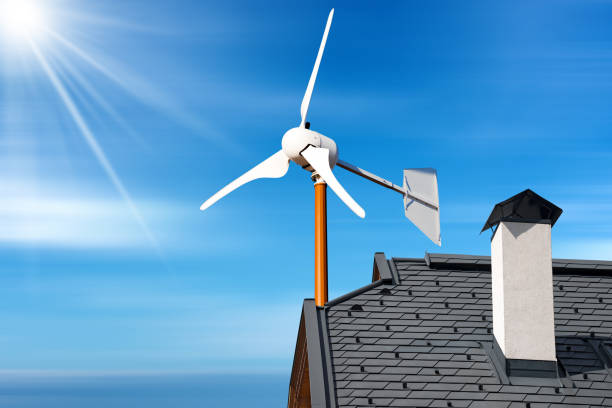
- Blade Design: The shape, length, and twist of the turbine blades significantly impact Cp. Aerodynamically efficient blades are designed to minimize drag and turbulence, maximizing energy extraction.
- Wind Direction: Turbines are most efficient when the wind hits the blades head-on. Changes in wind direction require the turbine to adjust its orientation to maintain optimal Cp.
- Tip Speed Ratio (TSR): TSR, the ratio of the blade tip speed to the wind speed, affects Cp. Each turbine has an optimal TSR at which it operates most efficiently.
- Pitch Control: Modern turbines often feature pitch control systems that allow the angle of the blades to be adjusted. Optimizing blade pitch according to wind conditions enhances efficiency.
Understanding these factors and their interplay is crucial for engineers and researchers working to improve turbine design. By maximizing Cp, wind energy systems can capture more energy from the wind, enhancing overall efficiency and contributing to a more sustainable energy future.
Calculating Area and Rotor Swept Area
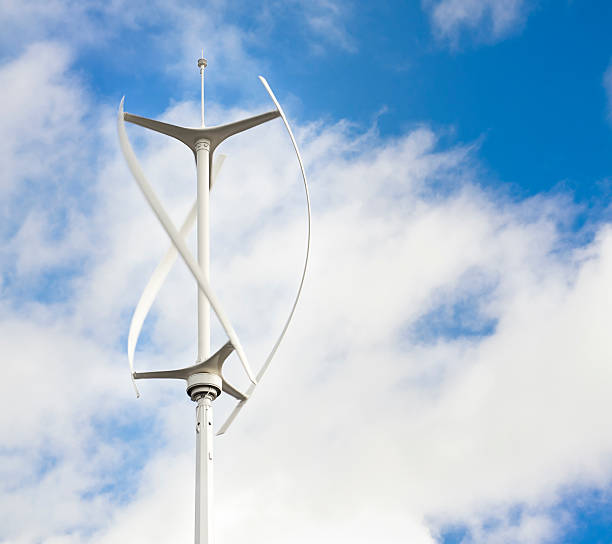
The area over which the wind turbine’s blades sweep, often referred to as the rotor swept area (A), is a fundamental parameter in wind energy output calculations. This area represents the circular space covered by the rotating blades as they capture the kinetic energy from the wind. Understanding how to calculate and optimize this area is essential for maximizing a wind turbine’s energy output.
Explanation of Rotor Swept Area (A):
The rotor swept area (A) is calculated using the formula:
A=π×(Blade Length)2
Where:
- π (pi) is a mathematical constant approximately equal to 3.14159.
- Blade Length is the length of one turbine blade from the rotor hub to the tip.
This formula calculates the circular area covered by a single blade. Since most modern wind turbines have multiple blades, the total rotor swept area is determined by multiplying the area of one blade by the number of blades.
Total Rotor Swept Area=A×Number of Blades
Importance of Swept Area in Energy Production:
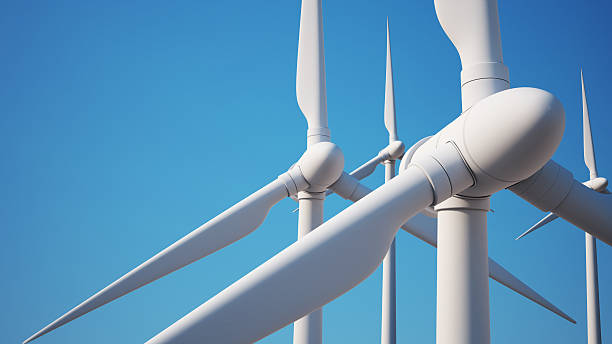
The rotor swept area plays a critical role in wind energy production for several reasons:
- Capture Efficiency: A larger swept area allows the turbine to capture more wind energy. Wind passing through a larger area means more air particles are interacting with the blades, resulting in higher energy extraction.
- Optimal Utilization: Wind turbines are often spaced apart in wind farms to prevent interference between neighboring turbines. Maximizing the swept area ensures efficient use of available land while harnessing as much wind energy as possible.
- Scaling Effects: Larger turbines with larger rotor diameters have higher energy production potential. By increasing the rotor swept area, wind farm operators can enhance the overall energy output and efficiency of their installations.
By accurately calculating and optimizing the rotor swept area, engineers can design wind turbines that are both efficient and economical. This optimization process is vital for the continuous advancement of wind energy technology, contributing to the global efforts in transitioning towards a sustainable energy future.
Considering Real-World Factors
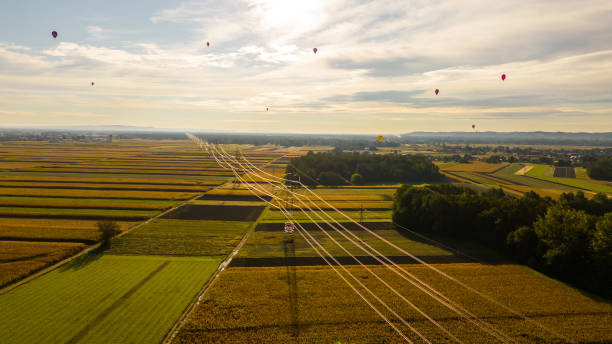
Wind energy output calculations, while based on fundamental principles and formulas, face various challenges and real-world factors that can significantly impact their accuracy. As wind energy continues to play a crucial role in our transition to sustainable power sources, it’s imperative to account for these complexities in both design and operation. Let’s explore some of the critical real-world factors that influence wind energy output calculations:
Downtime and Maintenance:
- Challenge: Wind turbines require regular maintenance and are occasionally taken offline for repairs, leading to downtime.
- Impact: Downtime reduces the overall energy production capacity of a wind farm, affecting the calculated output compared to theoretical values.
Environmental Factors:
- Challenge: Temperature, humidity, and altitude can affect air density, which, in turn, impacts wind energy output.
- Impact: Wind energy output calculations often need to consider variations in air density to provide accurate predictions of power generation in different weather conditions and locations.
Wind Turbine Performance:
- Challenge: Turbine performance can degrade over time due to wear and tear, leading to reduced efficiency.
- Impact: Lower efficiency means the turbine captures less energy from the wind, directly affecting the calculated output.
Wind Direction Variability:
- Challenge: Wind doesn’t always blow from a consistent direction; it can vary, affecting the angle at which the turbine blades intercept the wind.
- Impact: Changes in wind direction require the turbine to adjust its orientation, influencing the efficiency and, consequently, the energy output.
Turbulence and Wake Effects:
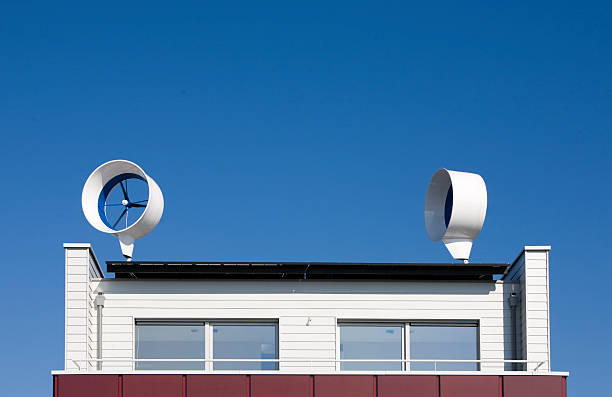
- Challenge: Turbulence caused by nearby obstacles or other wind turbines, as well as wake effects from upstream turbines, can reduce the efficiency of downstream turbines.
- Impact: Turbulence and wake effects lead to uneven wind distribution on the turbine blades, affecting their ability to capture energy efficiently.
Addressing these real-world challenges requires advanced modeling techniques, accurate data analysis, and continuous research and development efforts in wind energy technology. Engineers and scientists work collaboratively to improve turbine designs, enhance predictive algorithms, and optimize wind farm layouts to mitigate these factors and maximize energy output.
Conclusion: Harnessing the Power of Precision in Wind Energy Output Calculations
In the quest for a sustainable energy future, wind power stands as a beacon of hope, offering abundant clean energy potential. However, realizing this potential hinges on our ability to accurately predict and optimize wind energy output. Our exploration into wind energy output calculations has revealed the intricate science behind this process, encompassing variables like wind speed, turbine efficiency, rotor swept area, and real-world challenges.
Through precise calculations and innovative solutions, the wind energy industry continues to overcome hurdles, from variable terrains to offshore complexities. Advanced simulations, remote sensing technologies, predictive maintenance algorithms, and adaptive control systems have become indispensable tools, enabling engineers and researchers to harness the power of the wind more efficiently than ever before.
Key Insights:
- Wind Speed Matters: Wind speed, especially when raised to the power of three in the energy output equation, underscores the critical importance of accurate wind speed measurement and prediction.
- Turbine Efficiency is Key: Maximizing turbine efficiency, represented by the power coefficient (Cp), ensures optimal energy conversion from wind to electricity.
- Rotor Swept Area Optimization: Calculating and optimizing the rotor swept area is fundamental to capturing as much wind energy as possible.
- Real-World Challenges: Addressing real-world factors such as downtime, environmental variations, and wind direction fluctuations requires advanced technologies and adaptive strategies.
- Innovation and Collaboration: The synergy of innovation, data-driven insights, and interdisciplinary collaboration fuels the wind energy sector’s progress.
As we move forward, accurate wind energy output calculations will continue to shape the design, implementation, and operation of wind farms worldwide. They not only enhance energy production but also contribute significantly to mitigating climate change and reducing our dependence on fossil fuels.
In our pursuit of a sustainable future, the precision in wind energy output calculations becomes not just a scientific endeavor but a moral imperative. By refining our understanding, embracing emerging technologies, and fostering global collaboration, we can amplify the impact of wind energy, ushering in an era where clean, renewable power drives our world toward a greener, more sustainable tomorrow.